The Earth's ionosphere is that part of the high altitude
atmosphere, starting at about 90 km, which is strongly ionised.
Electrons are stripped off the gas molecules, resulting in ions, by
the ultra-violet radiation
(1 -10 x 10-8 m) of the Sun as well
as incident X-rays. The mix of positively and negatively charged
ions, negative electrons and neutral gas is called a plasma, which
is the most common state of the universe. The most abundant gas
molecules are molecular oxygen (O2) and nitrogen
(N2) below 200 km, atomic oxygen (O) above
200 km, and hydrogen (H) and Helium (He) above 600 km
altitude. Although less than 1% of the upper atmosphere becomes
ionised the charged particles make the gas electrically conducting,
which completely changes its characteristics. The ionosphere can
carry electrical currents as well as reflect, deflect and scatter
radio waves. It received its name from Sir Robert Watson-Watt in
only 1926, although Carl Friedrich Gauss had already speculated
about its existence in 1839.
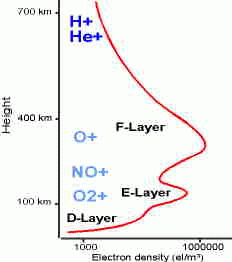 Figure 1: A typical electron density altitude profile,
the most important ions, and the various ionospheric layers.
The ionosphere is divided into different layers according to the
electron density present, which is always equal to the ion density.
Figure 1 shows a schematic of the different nomenclature, a typical
electron density profile and which ions dominate with altitude. Sir
Edward Appleton named the (E)lectrical-Layer in 1927 after Guglielmo
Marconi showed, during communication experiments in 1901, that radio
waves between Europe and America had to be bouncing off an
electrically conducting layer at around 100 - 150 km
altitude. Subsequently, other layers were discovered which simply
received the names D- and F-layers. The D-layer is particularly
complicated with more than 50 chemical reactions present. The
F-layer electron density can exceed 1012 m-3,
especially at high latitudes where high energy electrons originating
from the sun can impact onto the upper atmosphere causing additional
ionisation. The structure of the ionosphere is a balance between
solar production and the destructive processes of recombination. The
electron density undergoes a strong daily variation, especially at
sunrise and sunset, as well as annual fluctuations. The sun has an
11-year activity cycle, which is witnessed by the number of sunspots
on its surface. Figure 2 shows the number of sunspots from 1994
until the present (blue line) and the prediction for the next 7
years (red line). The next maximum is in 2001 where additional solar
X-ray radiation will increase the level of ionisation of the
ionosphere, thereby changing its radio wave propagation
characteristics.
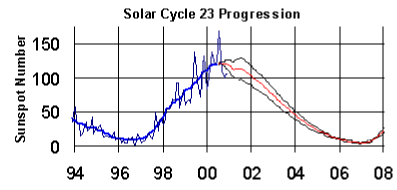 Figure 2: Number of sunspots versus time (blue line).
The red line is the prediction for the remaining part of the 11-year
cycle.
The aurora has long fascinated mankind (See Figure 3).
This natural optical phenomenon was often interpreted as warnings
from God in the 16th and 17th centuries or as signals of impending
disaster and war (See Figure 4).
Although early Greek and Roman philosophers such as Aristoteles and
Seneca theorised on the phenomenon of aurora, it was only in the
18th century that any serious interpretation was attempted. Many
explanations revolved around the reflection of sunlight by ice
crystals, clouds or atmospheric gases. Only after the spectral
measurements of Angström in 1867 did it become clear that the aurora
was caused by optical emissions of the atmospheric gases themselves.
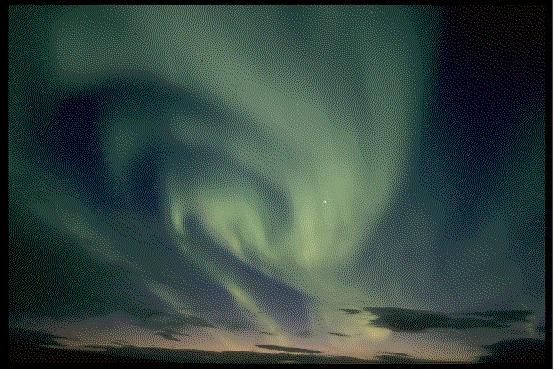
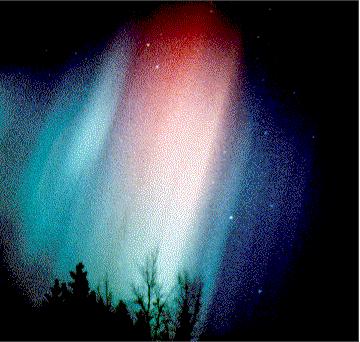 Figure 3: The colourful aurora. Top panel: A
common green aurora with some clouds and sunset in the background.
Bottom panel: The human eye's poor colour sensitivity make the red
and blue colours difficult to see. The bright spots are stars.
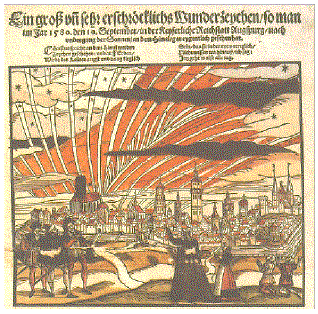 Figure 4: Pamphlet from 1580 depicting an
aurora over Augsburg, Germany.
What causes the aurora? Besides the heat and light emitted by the
sun, large quantities of charged particles blow off the sun's
surface to form the interplanetary solar wind. The solar wind
consists mainly of protons and electrons moving at ultra-sonic
speeds of 400 - 800 km/s (more than a million miles per
hour). The Earth is surrounded by its magnetic field in space,
called the magnetosphere, which forms a barrier to the solar wind.
Without the solar wind, the magnetic field lines of the Earth would
be symmetric and similar to those of a bar magnet. However, the
solar wind pressure strongly compresses the magnetosphere on the
dayside and draws it out into an extremely long tail on the
nightside of the Earth (See Figure 5).
Since the charged particles of the solar wind can not cross the
Earth's magnetic field lines, they flow around the magnetosphere
similar to water around a rock in a river. This forms a standing
shock wave in space upstream of the Earth, called the bow shock,
much like the boom of an aircraft breaking the sound barrier (See Figure 5).
The magnetospheric tail flaps in space much like a flag in the wind.
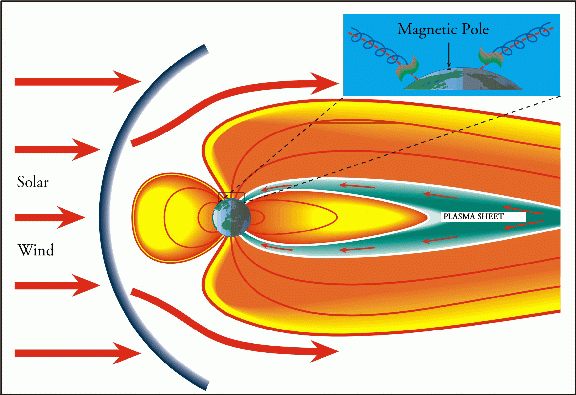 Figure 5: A schematic showing the solar wind,
bow shock, planet Earth, the magnetosphere and the plasmasheet. The
inset illustrates how electrons spiralling down magnetic field lines
may energise the atmospheric gases to emit light.
Through complicated and not completely understood processes,
electrons out of the solar wind are able to diffuse into
magnetospheric tail and form a reservoir called the plasma sheet.
The magnetosphere and the solar wind form an enormous electrical
dynamo where large and complicated electrical currents flow. One
component of these currents is carried by the electrons in the
plasma sheet which can descend along magnetic field lines along
spiral paths (See Figure 5).
Should these electrons descend down to altitudes of 100 -
300 km, they may collide with the atmospheric gas causing it to
glow. This, very basically, is the cause of aurora. Note that the
plasma sheet is only connected to magnetic field lines from the
Earth's polar regions on the nightside, which explains why the
aurora is mostly seen only at high latitudes at night. However,
dayside aurora also exist because solar wind particles have direct
access to the Earth's atmosphere via the cusp regions, which are
formed by the divide between magnetic field lines bent towards and
away from the sun (See Figure 5).
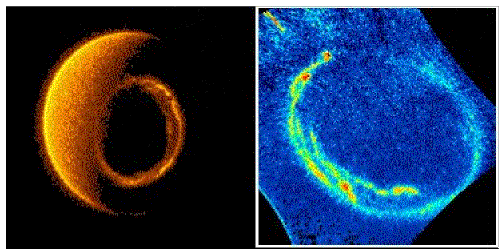 Figure 6: Satellite images of the auroral
oval. Left panel: A Dynamics Explorer image from 21000 km
altitude. The bright crescent is the sunlit dayside. Right panel: A
Viking image from 6000 km altitude taken at ultraviolet
wavelengths. The Earth's magnetic pole is in the center of the
ovals.
Since the magnetosphere is 3 dimensional, the regions of frequent
aurorae form an oval centered about the magnetic poles of the Earth
(See Figure 6).
During periods of high solar activity, especially around solar
maximum, massive explosions in the sun's atmosphere result in
Coronal Mass Ejections (CMEs). The energy released
(~1013 kg with speeds of 500 - 2000 km/s)
is so great that the earth's magnetosphere becomes sufficiently
deformed that the plasma sheet becomes connected to lower latitudes.
Hence, the auroral oval expands to such an extent that aurorae can
even be witnessed over the UK. Such events have negative
consequences for satellites and communication links. The colours
of the aurora depend on the chemical composition of the atmosphere
with altitude and the energy of the precipitating electrons. Higher
energies mean a deeper penetration of the atmosphere with a
corresponding change in composition. The typical colours of the
aurora are green (557.7 nm) and red (630 nm) from atomic
oxygen (O) and blue (391.4 and 427.8 nm) from molecular
nitrogen (N2) (See Figure 3).
Many other emissions also occur outside the visible wavelengths.
Auroral structures take on many shapes and movements. These result
from the changing spatial arrangement of the precipitating electrons
coming out of the plasma sheet. By studying the various aspects of
the aurora, much information can be gleened about space plasma
processes occurring out in the magnetosphere.
|